Research
Research themes and technologies
The research in the Allison Lab is primarily interested in how proteins do their jobs, the myriad of structural forms and interactions that affect their functioning, and by using structure-function relationships as a scaffold, we are interested in how this function can be tuned to yield desirable functional features. This involves studying both soluble and membrane proteins, with a particular emphasis on studying enzymes. Alongside the proteinaceous research, by studying the fundamentals of the experimental techniques we commonly use, and developing supporting software to expedite and enable new analyses, we try to uncover new and enabling methodologies.
A central technique and interest in our research is native mass spectrometry. Our laboratory hosts the New Zealand Centre for Native Mass Spectrometry, which is equipped with a Waters Synapt XS ion mobility-mass spectrometer. This instrument has ETD and a 32k quadrupole, and is configured for offline nano-electrospray ionisation, for which we fabricate custom glass capillary emitters. We also have a Waters ACQUITY UPLC M-Class that enables LC-MS workflows.
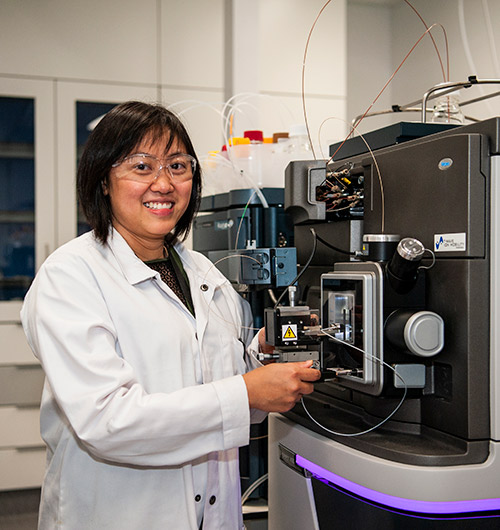
Research outputs
Software
See the Resources page for some of the software we develop.
Protein crystal structures
The protein models we create through protein crystallography (neat videos explaining the technique (Part 1, Part 2) from Elspeth Garman, University of Oxford) are all deposited in the Protein Data Bank. View all our structures in the Protein Data Bank or follow the individually linked PDB codes below to view each structure.
8F8F, 8F8K, 8F8L, 8PA0, 8RHA, 5DS2, 5DS1, 5J6F, 5EXG, 5EC5, 4NH2, 4IXX, 4HSN, 4HSO, 4JTE, 4JTF, 4JTG, 4JTH, 4JTI, 4JTJ, 4JTK, 4JTL, 4GRS, 3STC, 3STE, 3STF, 3STG, 3QPY, 3QPZ, 3QQ0, 3QQ1.
Publications
- Newton-Vesty, M.C., Currie, M.J., Davies, J.S., Panjikar, S., Sethi, A., Whitten, A.E., Tillett, Z.D., Wood, D.M., Wright, J.D., Love, M.J., Allison, T.M., Jamieson, S.A., Mace, P.D., North, R.A., Dobson, R.C.J. (2024) On the function of TRAP substrate-binding proteins: the isethionate-specific binding protein IseP. Biochem. J. 481 (24), 1901–1920.
- Feast, S., Titterington, J., Hoang, V.A., Allison, T.M., Fee, C., Nazmi, A.R. (2024) Purification of his-tagged proteins using printed monolith adsorption columns. J. Chromatogr. A 1733, 465216.
- Eade, L., Sullivan, M.P., Allison, T.M., Goldstone, D.C., Hartinger, C.G. (2024) Not All Binding Sites Are Equal: Site Determination and Folding State Analysis of Gas-Phase Protein-Metallodrug Adducts. Chem. Eur. J. 30, e202400268.
- Stanborough, T., Ho, N.A.T., Bulloch, E.M.M., Bashiri, G., Dawes, S.S., Akazong, E.W., Titterington, J., Allison, T.M., Jiao, W., Johnston, J.M. (2023) Allosteric inhibition of Staphylococcus aureus MenD by 1,4-dihydroxy naphthoic acid: a feedback inhibition mechanism of the menaquinone biosynthesis pathway. Phil. Trans. R. Soc. B 378 20220035.
- Saluri, M., Leppert, A., Gese, G.V., Sahin, C., Lama, D., Kaldmäe, M., Chen, G., Elofsson, A., Allison, T.M., Arsenian-Henriksson, M., Johansson, J., Lane, D.P., Hällberg, B.M., Landreh, M. (2023) A “grappling hook” interaction connects self-assembly and chaperone activity of Nucleophosmin 1. Proc. Natl. Acad. Sci. U.S.A. Nexus 2, pgac303.
- Given, F.M., Moran, F., Johns, A.S., Titterington, J.A., Allison, T. M., Crittenden, D.L., Johnston, J.M. (2022) The structure of His-tagged Geobacillus stearothermophilus purine nucleoside phosphorylase reveals a 'spanner in the works'. Acta Crystallogr. F. Struct. Biol. Commun. 78 416–422.
- Allison, T. M., Degiacomi, M.T., Marklund, E.G., Jovine, L., Elofsson, A., Benesch, J.L.P., Landreh, M. (2022) Complementing machine learning-based structure predictions with native mass spectrometry. Protein Sci. 31 e4333.
- Yen, H., Abramsson, M.L., Agasid, M.T., Lama, D., Gault, J., Liko, I., Kaldmäe, M., Saluri, M., Qureshi, A.A., Suades, A., Drew, D., Degiacomi, M.T., Marklund, E.G., Allison, T. M., Robinson, C.V., Landreh, M. (2022) Electrospray ionization of native membrane proteins proceeds via a charge equilibration step. RSC Adv. 12 9671–9680.
- Abramsson, M.L., Sahin, C., Hopper, J.T.S., Branca, R.M.M., Danielsson, J., Xu, M., Chandler, S.A., Österlund, N., Ilag, L.L., Leppert, A., Costeira-Paulo, J., Lang, L., Teilum, K., Laganowsky, A., Benesch, J.L.P., Oliveberg, M., Robinson, C.V., Marklund, E.G., Allison, T. M., Winther, J.R., Landreh, M. (2021) Charge engineering reveals the roles of ionizable side chains in electrospray ionization mass spectrometry. JACS Au 1 2385–2393.
- Sahin, C., Österlund, N., Leppert, A., Johansson, J., Marklund, E. G., Benesch, J. L. P., Ilag, L. L., Allison, T. M., Landreh, M. (2021) Ion mobility-mass spectrometry shows stepwise protein unfolding under alkaline conditions. Chem. Commun. 57 1450–1453.
- Allison, T. M., Barran, P., Cianferani, S., Degiacomi, M. T., Gabelica, V., Grandori, R., Marklund, E. G., Menneteau, T., Migas, L. G., Politis, A., Sharon, M., Sobott, F., Thalassinos, K., Benesch, J. L. P. (2020) Computational Strategies and Challenges for Using Native Ion Mobility Mass Spectrometry in Biophysics and Structural Biology. Anal. Chem. 92 10872–10880.
- Landreh, M., Shahin, C., Gault, J., Sadeghi, S., Drum, C. L., Uzdavinys, P., Drew, D., Allison, T. M., Degiacomi, M. T., Marklund, E. G. (2020) Predicting the Shapes of Protein Complexes through Collision Cross Section Measurements and Database Searches. Anal. Chem. 92 12297–12303.
- Allison, T. M., Barran, P., Benesch, J. L. P., Cianferani, S., Degiacomi, M. T., Gabelica, V., Grandori, R., Marklund, E. G., Menneteau, T., Migas, L. G., Politis, A., Sharon, M., Sobott, F., Thalassinos, K. (2020) Software Requirements for the Analysis and Interpretation of Native Ion Mobility Mass Spectrometry Data. Anal. Chem. 92 10881–10890.
- Bolla, J. R., Corey, R. A., Sahin, C., Gault, J., Hummer, A., Hopper, J. T. S., Lane, D. P., Drew, D., Allison, T. M., Stansfeld, P. J., Robinson, C. V., Landreh, M. (2020) A mass spectrometry-based approach to distinguish annular and specific lipid binding to membrane proteins. Angew. Chem. Int. Ed. Engl. 59 3523–3528.
- Allison, T. M., Agasid, M. T. (2020) Native Protein Mass Spectrometry. Protein Nanotechnology. Methods in Molecular Biology v.2073.
- Kaldmäe, M., Österlund, N., Lianoudaki, D., Sahin, C., Bergman, P., Nyman, T., Kronqvist, N., Ilag, L. L., Allison, T. M., Marklund, E. G., Landreh, M. (2019) Gas-phase collisions with trimethylamine-N-oxide enable activation-controlled protein ion charge reduction. J. Am. Soc. Mass Spectrom. 30 1385–1388.
- Collier, M. P., Alderson, T. R., de Villiers, C. P., Nicholls, D., Gastall, H. Y., Allison, T. M., Degiacomi, M. T., Jiang, H., Mlynek, G., Fürst, D. O., van der Ven, P. F. M., Djinovic-Carugo, K., Baldwin, A. J., Watkins, H., Gehmlich, K., Benesch, J. L. P. (2019) HspB1 phosphorylation regulates its intramolecular dynamics and mechanosensitive molecular chaperone interaction with filamin C. Sci. Adv. 5, eaav8421.
- Allison, T. M., Bechara, C. (2019) Structural mass spectrometry comes of age: new insight into protein structure, function and interactions. Biochem. Soc. Trans. 47, 317–327.
- Allison, T. M., Landreh, M. (2019) Ion Mobility in Structural Biology. Comprehensive Analytical Chemistry 83, 161–195.
- Gault, J., Lianoudaki, D., Kaldmäe, M., Kronqvist, N., Rising, A., Johansson, J., Lohkamp, B., Laín, S., Allison, T. M., Lane, D. P., Marklund, E. G., Landreh, M. (2018) Mass spectrometry reveals the direct action of a chemical chaperone. J. Phys. Chem. Lett. 9 4082–4086.
- Yewdall, N. A., Allison, T. M., Pearce, F. G., Robinson, C. V., Gerrard, J. A. (2018) Self-assembly of toroidal proteins explored using native mass spectrometry. Chem. Sci. 9 6099–6106.
- Liko, I., Degiacomi, M. T., Lee, S., Newport, T. D., Gault, J., Reading, E., Hopper, J. T. S., Housden, N. G., White, P., Colledge, M., Sula, A., Wallace, B. A., Kleanthous, C., Stansfeld, P. J., Bayley, H., Benesch, J. L. P., Allison, T. M., Robinson, C. V. (2018) Lipid binding attenuates channel closure of the outer membrane protein OmpF. Proc. Natl. Acad. Sci. U.S.A. 115 6691–6696.
- Hochberg, G. K. A., Shepherd, D. A., Marklund, E. G., Santhanagoplan, I., Degiacomi, M. T., Laganowksy, A., Allison, T. M., Basha, E., Marty, M. T., Galpin, M. R., Struwe, W. B., Baldwin, A. J., Vierling, E., Benesch, J. L. P. (2018) Structural principles that enable oligomeric small heat-shock protein paralogs to evolve distinct functions. Science 359 930–935.
- Bolla, J. R., Sauer, J. B., Wu, D., Mehmood, S., Allison, T. M., Robinson, C. V. (2018) Direct observation of the influence of cardiolipin and antibiotics on lipid II binding to MurJ. Nat. Chem. 10 363–371.
- Yen, H., Hopper, J. T. S., Liko, I., Allison, T. M., Zhu, Y., Wang, D., Stegmann, M., Mohammed, S., Wu, B., Robinson, C. V. (2017) Ligand binding to a G protein-coupled receptor captured in a mass spectrometer. Sci. Adv. 3 e1701016.
- Hopper, J. T. S., Ambrose, S., Grant, O. C., Krumm, S., Allison, T. M., Degiacomi, M. T., Tully, M. D., Pritchard, L. K., Ozorowski, G., Ward, A. B., Crispin, M., Doores, K. J., Woods, R. J., Benesch, J. L. P., Robinson, C. V., Struwe, W. B. (2017) The tetrameric plant lectin BanLec neutralises HIV through bidentate binding to specific viral glycans. Structure 25 773–782.
- Liko, I., Allison, T. M., Hopper, J. T. S., Robinson, C. V. (2016) Mass spectrometry guided structural biology. Curr. Opin. Struct. Biol. 40 136–144.
- Nazmi, A. R., Lang, E. J. M., Bai, Y, Allison, T. M., Othman, M. H., Panjikar, S., Arcus, V. L., Parker, E. J. (2016) Interdomain conformational changes provide allosteric regulation en route to chorismate. J. Biol. Chem. 291 21836–21847.
- Liko, I., Hopper, J. T. S., Allison, T. M., Benesch, J. L. P., Robinson, C. V. (2016) Negative ions enhance survival of membrane protein complexes. J. Am. Soc. Mass Spectrom. 27 1099–1104.
- *Allison T. M., *Landreh, M., Benesch, J. L. P., Robinson, C. V. (2016) Low charge and reduced mobility of membrane protein complexes has implications for calibration of collision cross section measurements. Anal. Chem. 88 5879–5884.
- Podobnik, M., Savory, P., Rojko, N., Kisovec, M., Wood, N., Hambley, R., Pugh, J., Wallace, J., McNeill, L., Bruce, M., Liko, I., Allison, T. M., Mehmood, S., Yilmaz, N., Kobayashi, T., Gilbert, R. J. C., Robinson, C. V., Jayasinghe, L., Anderluh, G. (2016) Crystal structure of an invertebrate cytolysin pore reveals unique properties and mechanism of assembly. Nat. Commun. 7, 11598.
- Allison T. M., Reading, E., Liko, I., Baldwin, A. J., Laganowsky, A., Robinson C. V. (2015) Quantifying the stabilizing effects of protein-ligand interactions in the gas phase. Nat. Commun. 6, 8551.
- *Reading, E., *Liko, I., Allison T. M., Benesch, J. L. P., Laganowsky, A., Robinson, C. V. (2015) The role of the detergent micelle in preserving the structure of membrane proteins in the gas phase. Angew. Chem. Int. Ed. Engl. 54, 4577–4581.
- *Mehmood, S., *Allison T. M., Robinson C. V. (2015) Mass spectrometry of protein complexes: from origins to applications. Annu. Rev. Phys. Chem. 66, 453–474.
- Mehmood, S., Marcoux, J., Hopper, J. T, Allison, T. M., Liko, I., Borysik, A. J., Robinson, C. V. (2014) Charge reduction stabilizes intact membrane protein complexes for mass spectrometry. J. Am. Chem. Soc. 136, 17010–17012.
- *Laganowsky, A., *Reading, E., Allison, T. M., Ulmschneider, M. B., Degiacomi, M. T., Baldwin A. J., Robinson, C. V. (2014) Membrane proteins bind lipids selectively to modulate their structure and function. Nature 510, 172–175.
- Cross, P. J., Pietersma, A. L., Allison, T. M., and Parker, E. J. (2013) Neisseria meningitidis expresses a single 3-deoxy-d-arabino-heptulosonate 7-phosphate synthase that is inhibited primarily by phenylalanine. Protein Science 22, 1087–1099.
- Allison, T. M., Cochrane, F. C., Jameson, G. B., and Parker, E. J. (2013) Examining the role of intersubunit contacts in catalysis by 3-deoxy-d-manno-octulosonate 8-phosphate synthase. Biochemistry 52, 4676–4686.
- Cross, P. J., Allison, T. M., Dobson, R. C. J., Jameson, G. B., and Parker, E. J. (2013) Engineering allosteric control to an unregulated enzyme by transfer of a regulatory domain. Proc. Natl. Acad. Sci. U.S.A. 110 2111–2116.
- Allison, T. M., Hutton, R. D., Wanting, J., Gloyne, B. J., Nimmo, E. B., Jameson, G. B., and Parker, E. J. (2011) An extended b7a7 substrate-binding loop is essential for efficient catalysis by 3-deoxy-d- manno-octulosonate 8-phosphate synthase. Biochemistry 50, 9318–9327.
- Allison, T. M., Hutton, R. D., Cochrane, F. C., Yeoman, J. A., Jameson, G. B., and Parker, E.J. (2011) Targeting the role of a key conserved motif for substrate selection and catalysis by 3- deoxy-d-manno-octulosonate 8-phosphate synthase. Biochemistry 50, 3686–3695.
- Allison, T. M., Yeoman, J. A., Hutton, R. D., Cochrane, F. C., Jameson, G. B., and Parker, E. J. (2010) Specificity and mutational analysis of the metal-dependent 3-deoxy-d-manno-octulosonate 8-phosphate synthase from Acidithiobacillus ferrooxidans. Biochim. Biophys. Acta, Proteins Proteomics 1804, 1526–1536.
* These authors contributed equally